
Seasonal Monitoring of Microclimate Parameters of Honeybee (Apis mellifera) Colonies in Relation to External Climatic Conditions in South Korea
Abstract
Accelerating climate change has increasingly challenged apicultural farms in managed honeybee (Apis mellifera) colonies. Although various management strategies are being explored to adapt to extreme climate events, fundamental data on the domestic environmental conditions remain limited. This study aims to document annual variations in the internal microclimate of honeybee hives and evaluate the impact of external climatic conditions on the hives’ internal environment. Temperature and relative humidity sensors were installed both inside and outside the hives across 10 apiaries in 9 regions of the Republic of Korea. These weather conditions(temperature and relative humidity) were monitored for a period of one year. Results indicated that internal hive temperatures ranged from -8.2°C to 49.6°C, while average internal relative humidity levels ranged from 16.4% to 97.8%. Comparisons by hive material revealed that EPP (Expanded polypropylene) hives demonstrated more stable internal temperature and relative humidity patterns in response to fluctuations of external temperature and relative humidity. Additionally, external temperature and relative humidity were positively correlated with the internal hive microclimate. These findings provide foundational insights to inform honeybee management practices under domestic climate conditions.
Keywords:
Managed pollinator, Temperature, Relative humidity, EPP, SARIMAX modelINTRODUCTION
Rapid climate change has significantly affected the composition of local native species, impacting both plants and animals. These changes have led to alterations in the ecosystems of affected regions (Walther, 2010; Burkle and Alarcon, 2011; Peck, 2011; Pauls et al., 2013; Bradley et al., 2024). Beyond native species, human-managed organisms, such as crops and livestock, are also profoundly impacted, often resulting to economic losses (Zilberman et al., 2004; Nelson et al., 2014; Altieri et al., 2015; Monteleone et al., 2023). Consequently, climate change has continued to harm agriculture (Rosenzweig et al., 2002; Rusmayandi et al., 2023). Although various solutions have been proposed (Howden et al., 2007; Davies et al., 2009; Agovino et al., 2019), they remain insufficient.
Most insects that interact with crops in agroecosystems are also greatly affected by climate change (Skendzic et al., 2021). Among these, pollinators play a critical role by providing ecosystem services that support both wild and cultivated plants. Shifts in pollinator diversity and abundance can significantly affect pollination services, with broad implications on the surrounding flora (Giannini et al., 2017). Pollinators are generally classified into managed pollinators, such as honeybees (Apis spp.) and bumblebees (Bombus spp.), and wild pollinators, which inhabit natural ecosystems and provide essential pollination services for plants in nearby forests and agricultural areas (Garibaldi et al., 2014; Pecenka et al., 2023). In particular, wild pollinators interact closely with their habitats, and plant diversity in these environments can both influence and be influenced by their activities (Eeraerts, 2023). Recently, studies have focused on conserving and enhancing pollinator populations to improve the productivity of open-field crops (Feltham et al., 2015; Garibaldi et al., 2017; Aguilera et al., 2020). However, the increasing impacts of climate change continue to thwart efforts to maintain and manage these populations effectively.
Managed pollinators, such as honeybees (A. mellifera), are essential for honey production and crop pollination within controlled environments, creating significant economic value through industrial applications (Losey and Vaughan, 2006; Jung and Shin, 2022). Specifically, the honeybee is widely utilized across various crops, contributing substantial value to the agricultural sector (Yoon et al., 2021). Beekeeping products further bolster this contribution, positioning the honeybee as a key species within the industrial insect market (Resh and Carde, 2009; Hill, 2012). Nonetheless, the industry has recently suffered considerable economic losses due to colony declines caused by pests, pesticide exposure, climate change, and other external stressors (Hristov et al., 2020; de Jongh et al., 2022).
In response, international efforts are underway to collect foundational data by monitoring environmental factors such as internal hive temperature, relative humidity, and additional conditions affecting the health of honeybee colonies (Flores et al., 2019; Neov et al., 2019; Thoms et al., 2019). However, research on environmental monitoring within honeybee colonies, specifically adapted to domestic conditions, remains limited. Additionally, wooden and EPP materials are the most commonly used for beehives in South Korea (Lee et al., 2015). Wooden hives are relatively inexpensive but heavy, whereas EPP hives are lightweight yet more expensive. These differences in material properties may significantly influence the internal microclimate of beehives, which is a critical factor for the health and productivity of honeybee colonies (Cook et al., 2021). Despite this, the impact of hive material on the stability of internal temperature and relative humidity, particularly under varying climatic conditions, remains insufficiently studied.
This study aims to analyze annual patterns of temperature and relative humidity within honeybee colonies in response to external climate conditions. To achieve this, thermosensors were installed both inside and outside honeybee hives to continuously record these variables throughout the year. The resulting data were analyzed to evaluate changes in internal temperature and relative humidity as influenced by external climate factors, focusing specifically on the two widely utilized hive materials in South Korea: Epp and wood. Furthermore, the SARIMAX model was employed to quantify the impact of external climatic conditions on the stability of internal hive microclimates.
MATERIALS AND METHODS
1. Study site and insect
The experiment was conducted across 10 beekeeping farms located in nine regions within seven provinces in South Korea (Table 1). Three honeybee (A. mellifera) colonies were used per region. At each beekeeping farm, two wooden hives and one EPP (Expanded polypropylene) hive were selected for the experiment. The initial colony strength was standardized to an average of 4.3±1.3 frames per single brood chamber, and colonies were maintained in this single-chamber setup throughout the monitoring period. Sugar syrup and pollen patties were provided as needed based on the timing and colony strength, while pest and disease control was conducted according to standard practices.
2. Monitoring of temperature and relative humidity
Monitoring was conducted for approximately one year, from July 25, 2023, to July 1, 2024. The data recording interval was set to one hour. Sensors (SHT30-ARP-B, Sensirion AG, Zurich) for temperature and relative humidity monitoring were installed in three locations within the hive (Langstroth hive): the bottom, the side, and the center (positioned at the center of the comb). Temperature and relative humidity data were collected from Daeseong Hive company (Jeonju, South Korea).
3. Data analysis
The seasonal mean temperature and relative humidity between the external and internal environments of the beehive were compared using a t-test. A one-way ANOVA (95% confidence level) was conducted to compare the mean internal and external temperature and relative humidity of honeybee colonies across different experimental sites, followed by a post hoc test using Tukey’s HSD. Normality and homogeneity of variance were tested prior to performing analysis of variance. Levene’s test was conducted to examine differences in the variability of diurnal temperature and relative humidity fluctuations within the beehive according to the material. Subsequently, one-way ANOVA was used to test for differences in the means. The SARIMAX (Seasonal autoregressive integrated moving average with exogenous variables) model was used to evaluate the impact of external temperature and relative humidity on internal temperature and relative humidity changes in the beehive. The suitability of the evaluation model was assessed using fit indices such as AIC (Akaike information criterion) and BIC (Bayesian information criterion), and prediction errors were calculated using RMSE (Root mean squared error) and MAPE (Mean absolute percentage error). Additionally, the predictive accuracy of the SARIMAX model for internal temperature changes was evaluated by comparing the model-generated predictions with actual observed values. All analyses were performed using the R software Version 4.4.1 (R Core Team, 2023).
RESULTS
1. Annual microclimate monitoring of apiaries and beehives
Over one year, from July 2023 to July 2024, a total of 161,277 data points on temperature and relative humidity were collected across South Korea. The average internal hive temperature across all sites was 26.2±14.7℃, with an external average of 17.2±11.4℃. Average internal relative humidity was 74.3±9.9%, compared to an external average of 62.3±23.6%.
Significant seasonal variations were observed in both internal and external microclimate parameters (Table 2). Internal temperatures peaked during summer (33.8±4.3°C) and were lowest during winter (10.0±3.2°C), reflecting external climatic conditions. Similarly, internal relative humidity levels showed seasonal trends, with the highest levels recorded in summer (77.2±5.8%) and the lowest in winter (64.8±6.5%). Despite these variations, the internal microclimate demonstrated greater stability compared to external conditions, indicating that honeybee colonies effectively regulate their hive environments to mitigate the impact of seasonal climatic fluctuations.

Seasonal variations in internal and external temperature (Mean±SD ℃) and relative humidity (Mean±SD %) of honeybee (A. mellifera) hives
External temperatures ranged from -10.0℃ to 70.0℃, while external relative humidity varied from 0% to 100% (Table 3). The highest mean annual external temperature was recorded in Gimhae (20.1±11.3℃), and the lowest in Jangheung (13.4±14.7℃). The widest annual mean daily temperature range outside the hives was observed in Hoengseong1 (25.2±8.6℃), while the narrowest range was found in Namwon (10.6±5.5℃). Relative humidity followed a similar pattern, with the highest external annual mean in Namwon (71.2±13.8%) and the lowest in Jangheung (40.2±38.7%). Across all apiary sites, the internal temperature of the beehives ranged from a minimum of -8.2℃ to a maximum of 49.6℃, while the relative humidity ranged from a minimum of 16.4% to a maximum of 97.8% (Table 4). The highest annual mean internal temperature of the beehives was observed in Dangjin at 26.0±7.2℃, while the lowest was recorded in Sancheong at 19.1±11.4℃. The highest annual mean daily temperature range inside the beehives was observed in Hoengseong1 at 13.0±4.5℃, while the lowest was recorded in Yecheon at 5.2±2.2℃. The average internal relative humidity of the beehives was highest in Namwon at 78.9±5.9% and lowest in Hoengseong1 at 69.5±7.9%. The annual mean daily relative humidity range inside the beehives was highest in Hoengseong1 at 21.7±7.3% and lowest in Namwon at 9.9±5.4%.

External beehive temperature (℃) and relative humidity (%) data, including minimum, maximum, annual mean, and diurnal temperature range

Internal beehive temperature (℃) and relative humidity (%) data, including minimum, maximum, annual mean, and diurnal temperature range
The internal temperature of the beehives ranged from -8.2℃ to 49.6℃, while the relative humidity varied between 16.4% and 97.8% (Table 4). Regionally, Dangjin recorded the highest mean internal temperature (26.0±7.2℃), while Sancheong had the lowest (19.1±11.4℃). The widest daily temperature range was observed in Hoengseong1 (13.0±4.5℃), and the narrowest in Yecheon (5.2±2.2℃). Internal relative humidity was highest in Namwon (78.9±5.9%) and lowest in Hoengseong1 (69.5±7.9%).
2. Temperature and relative humidity variability by beehive material
Analysis of temperature variability by hive material revealed significant differences, with EPP hives showing more stable internal temperatures and lower diurnal fluctuations compared to wooden hives (F1, 5027=47.23, P<0.001; Fig. 1). Diurnal temperature fluctuations inside wooden hives were significantly higher than in EPP (F1, 5024=153.6, P<0.001). Similarly, diurnal relative humidity variability differed significantly between the materials, with EPP hives demonstrating lower fluctuations (F1, 5024=142.9, P<0.001; Fig. 2). Levene’s test confirmed a significant difference in diurnal relative humidity variability between EPP and wood (F1, 5024=25.70, P<0.001).
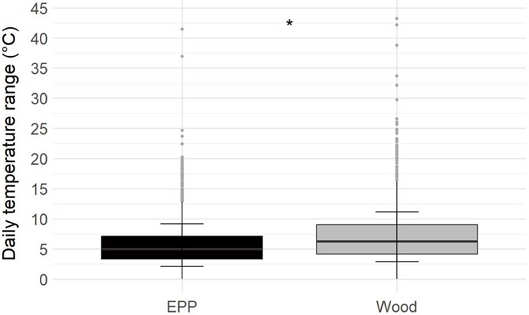
Temperature fluctuation range inside honeybee (A. mellifera) hives made of different materials (EPP and wood) during the monitoring period in South Korea. Error bars represent the standard deviation, and gray dots indicate outliers. Levene’s Test was used to assess the difference in variability of temperature fluctuations between the two materials (F1, 5024=47.23, P<0.001), and a one-way ANOVA was used to test for differences in mean fluctuation range (F1, 5024=153.6, P<0.001).
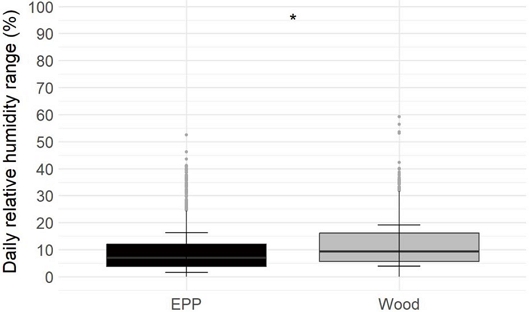
Relative humidity fluctuation range inside honeybee (A. mellifera) hives made of different materials (EPP and wood) during the monitoring period in South Korea. Error bars represent the standard deviation, and gray dots indicate outliers. Levene’s Test was used to assess the difference in variability of temperature fluctuations between the two materials (F1, 5024=25.70, P<0.001), and a one-way ANOVA was used to test for differences in mean fluctuation range (F1, 5024=142.9, P<0.001).
3. Influence of external climate on the internal microclimate in the beehive
The SARIMAX model analysis showed that both external temperature and relative humidity significantly influenced internal hive temperature (Table 5). The model estimated an external temperature coefficient of 0.3657, indicating that a 1℃ increase in external temperature is associated with an approximate 0.37℃ increase inside the hive. The coefficient for external relative humidity was 0.0331, suggesting that a 1% rise in external relative humidity results in an approximate 0.03℃ increase in internal temperature. Model performance metrics yielded an AIC of 825.6, BIC of 856.06, RMSE of 0.8008, and MAPE of 2.85%, indicating reliable prediction accuracy, with seasonal patterns closely aligning between observed and predicted values (Fig. 3).

SARIMAX model evaluating the effects of external temperature and relative humidity on beehive temperature and relative humidity in the beehive
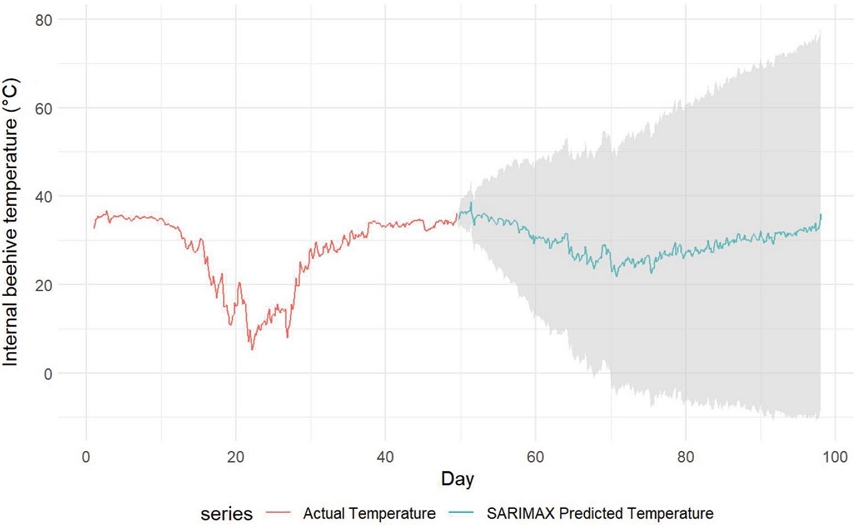
Prediction of internal beehive temperature using the SARIMAX model. The red line represents the actual measured internal temperature of the hive, while the cyan line shows the temperature predicted by the SARIMAX model. The gray area represents the prediction interval, visually indicating the model’s uncertainty.
Similarly, both external temperature and relative humidity significantly impacted internal hive relative humidity. The external temperature coefficient was 0.2431, suggesting a 1℃ increase in external temperature raises internal relative humidity by approximately 0.2431%. The external relative humidity coefficient was 0.0901, indicating that a 1% increase externally corresponds to approximately 0.0901% rise internally. Model fit metrics for relative humidity yielded an AIC of 1048.08, BIC of 1078.55, RMSE of 1.1208, and MAPE of 1.36%, with seasonal patterns of internal relative humidity aligning closely with actual observations (Fig. 4).
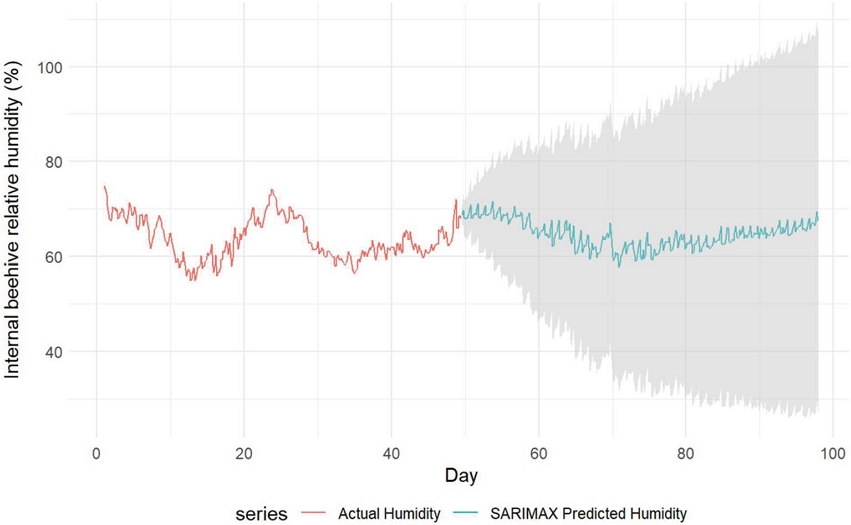
Prediction of internal beehive relative humidity using the SARIMAX model. The red line represents the actual measured internal temperature of the hive, while the cyan line shows the temperature predicted by the SARIMAX model. The gray area represents the prediction interval, visually indicating the model’s uncertainty.
DISCUSSION
Monitoring of internal and external temperature and relative humidity across 10 apiaries in South Korea over a one-year period showed that the internal temperature range of the hives varied from -8.2℃ to 49.6℃. The external temperature range was observed to range between -10℃ and 70℃, suggesting that honeybees likely expended significant energy on thermoregulatory behaviors to adapt to these harsh external climate conditions. Honeybees must maintain the central hive temperature at approximately 33-36℃, except during hibernation (Heldmaier, 1987; Humphrey and Dykes, 2008). However, when unexpected cold spells in spring or extreme heat waves in summer cause external temperatures to exceed this optimal range, honeybees are forced to expend greater energy than usual to regulate the hive temperature (Stabentheiner et al., 2010). According to Rodriguez-Vasquez et al. (2024), the acceptable temperature thresholds within colony are defined as 6-38℃. Exceeding this range can lead to severe metabolic damage in honeybees. Ultimately, this can adversely affect the entire colony, potentially leading to its losses. This suggests that, despite their microclimate regulation abilities, honeybees may find it increasingly difficult to survive and function under rapidly changing external climate conditions.
The average temperature and relative humidity inside and outside the hives showed significant differences across seasons. South Korea experiences four distinct seasons throughout the year, which contributed to notable variations in the external climate and the internal microclimate of the hives in each season. These differences are likely to have a substantial impact on honeybees (Knoll et al., 2020). To alleviate the stress caused by these climatic fluctuations, various management strategies are required.
The variability of internal hive microclimates differed across regions, showing a range of temperatures and relative humidity levels. Notably, in the same region, Hoengseong1 and Hoengseong2 exhibited similar annual average internal temperatures, but their annual average daily temperature ranges within the hives differed. This suggests that even within the same area, factors such as hive placement, surrounding structures, terrain, and the presence of nearby water sources can influence the external climate conditions impacting the hives (Bordier et al., 2017). For example, Hoengseong1 featured shelter facilities installed over the beehives, providing shade and some protection from direct environmental exposure, whereas Hoengseong2 lacked such facilities, leading to greater exposure to external climate variability. Supplementary studies on additional environmental factors, including investigations of surrounding topographic features and landscape are needed to better understand their impacts on hive microclimates.
The internal temperature and relative humidity of EPP and wooden hives exhibited different variability in response to external climate conditions. EPP hives maintained more stable internal temperatures and relative humidity levels compared to wooden hives. In particular, the smaller daily fluctuations in temperature and relative humidity within EPP hives indicate superior insulation properties of the EPP material. According to Cook et al. (2021), EPP hives can reduce heat loss by up to 77% compared to wooden hives of the same thickness, due to the lower thermal conductivity of EPP, which makes it less susceptible to external temperature fluctuations. Although domestic beekeepers initially expressed concerns over relative humidity management in EPP hives, despite their superior insulation compared to wooden hives, this study confirmed that EPP also demonstrated better stability in relative humidity. The use of EPP hives suggests a potential management strategy to alleviate environmental stress on honeybees caused by climate change and extreme weather events.
Assessment of the impact of external climate conditions on the internal microclimate of honeybee hives revealed that a 1℃ increase in external temperature corresponded to a 0.3657℃ increase in hive temperature, underscoring the substantial influence of external conditions on hive microclimate stability. These findings provide quantitative evidence that variations in external climate can significantly alter the internal hive environment, highlighting the necessity for refined management strategies. Such strategies may include targeted temperature control measures based on hive location and surrounding environmental factors to maintain optimal conditions for colony health. Aligned with findings by Ziegler et al. (2022), which highlight the strong association between climatic factors and honeybee colony productivity, the results of this study emphasize the critical role of microclimate management within the honeybee ecosystem. Ziegler et al. demonstrated that hive weight functions as an indirect indicator of colony size and foraging productivity, with external temperature exerting a significant influence on hive weight variability. These results suggest that fluctuations in external temperature may compel honeybees to expend additional energy to stabilize internal hive temperatures, potentially exerting adverse effects on colony health and overall productivity. Furthermore, a substantial body of research has indicated that climate change is likely to exert adverse effects on honeybee populations (Conte and Navajas, 2008; Cunningham et al., 2022; Zapata-Hernandez et al., 2024), highlighting the ongoing need for quantitative data to provide more robust support for these conclusions. By providing quantitative measurements of internal hive temperature fluctuations in response to external temperature increases, this study offers foundational data that may inform strategies for environmental adaptation and management of honeybee colonies under projected climate change scenarios.
In this study, we conducted year-round monitoring of external climate conditions and internal hive microclimate to evaluate the variability in internal temperature and relative humidity across different hive materials, as well as the influence of external climate on internal microclimate stability. These findings highlight the need for further research into the specific impacts of internal microclimate on honeybee behaviors and suggest investigating the long-term effects on colony health in relation to hive material and placement.
Acknowledgments
This research was funded by the Rural Development Administration (RDA) research project (PJ01595303).
References
-
Agovino, M., M. Casaccia, M. Ciommi, M. Ferrara and K. Marchesano. 2019. Agriculture, climate change and sustainability: The case of EU-28. Ecol. Indic. 105: 525-543.
[https://doi.org/10.1016/j.ecolind.2018.04.064]
-
Aguilera, G., T. Roslin, K. Miller, G. Tamburini, K. Birkhofer, B. Caballero-Lopez, S. A. Lindstrom, E. Ockinger, M. Rundlof, A. Rusch, H. G. Smith and R. Bommarco. 2020. Crop diversity benefits carabid and pollinator communities in landscapes with semi-natural habitats. J. Appl. Ecol. 57(11): 2170-2179.
[https://doi.org/10.1111/1365-2664.13712]
-
Altieril, M. A., C. I. Nicholls, A. Henao and M. A. Lana. 2015. Agroecology and the design of climate change-resilient farming systems. Agron. Sustain. Dev. 35: 869-890.
[https://doi.org/10.1007/s13593-015-0285-2]
-
Bordier, C., H. Dechatre, S. Suchail, M. Peruzzi, S. Soubeyrand, M. Pioz, M. Pelissier, D. Crauser, Y. L. Conte and C. Alaux. 2017. Colony adaptive response to simulated heat waves and consequences at the individual level in honeybees (Apis mellifera). Sci. Rep. 7(1): 3760.
[https://doi.org/10.1038/s41598-017-03944-x]
-
Bradley, B. A., E. M. Beaury, B. Gallardo, I. Ibanez, C. Jarnevich, T. L. Morelli, H. R. Sofaer, C. J. B. Sorte and M. Vila. 2024. Observed and potential range shifts of native and nonnative species with climate change. Annu. Rev. Ecol. Evol. Syst. 55: 23-40.
[https://doi.org/10.1146/annurev-ecolsys-102722-013135]
-
Burkle, L. A. and R. Alarcon. 2011. The future of plant-pollinator diversity: Understanding interaction networks across time, space, and global change. Am. J. Bot. 98(3): 528-538.
[https://doi.org/10.3732/ajb.1000391]
-
Conte, Y. L. and M. Navajas. 2008. Climate change: impact on honey bee populations and diseases. Rev. Sci. Tech. Off. Int. Epizoot. 27(2): 499-510.
[https://doi.org/10.20506/rst.27.2.1819]
-
Cook, D., A. Blackler, J. McGree and C. Hauxwell. 2021. Thermal impacts of apicultural practice and products on the honey bee colony. J. Econ. Entomol. 114(2): 538-546.
[https://doi.org/10.1093/jee/toab023]
-
Cunningham, M. M., L. Tran, C. G. McKee, R. O. Polo, T. Newman, L. Lansing, J. S. Griffiths, G. J. Bilodeau, M. Rott and M. M. Guarna. 2022. Honey bees as biomonitors of environmental contaminants, pathogens, and climate change. Ecol. Indic. 134: 108457.
[https://doi.org/10.1016/j.ecolind.2021.108457]
-
Davies, M., B. Guenther, J. Leavy, T. Mitchell and T. Tanner. 2009. Climate change adaptation, disaster risk reduction and social protection: Complementary roles in agriculture and rural growth? IDS Work. Pap. 320: 1-37.
[https://doi.org/10.1111/j.2040-0209.2009.00320_2.x]
-
de Jongh, E. J., S. L. Harper, S. S. Yamamoto, C. J. Wright, C. W. Wilkinson, S. Ghosh and S. J. G. Otto. 2022. One health, one hive: A scoping review of honey bees, climate change, pollutants, and antimicrobial resistance. PLoS One 17(2): e0242393.
[https://doi.org/10.1371/journal.pone.0242393]
-
Eeraerts, M. 2023. A minimum of 15% semi-natural habitat facilitates adequate wild pollinator visitation to a pollinator-dependent crop. Biol. Conserv. 278: 109887.
[https://doi.org/10.1016/j.biocon.2022.109887]
-
Feltham, H., K. Park, J. Minderman and D. Goulson. 2015. Experimental evidence that wildflower strips increase pollinator visits to crops. Ecol. Evol. 5(16): 3523-3530.
[https://doi.org/10.1002/ece3.1444]
-
Flores, J. M., S. Gil-Lebrero, V. Gamiz, M. I. Rodriguez, M. A. Ortiz and F. J. Quiles. 2019. Effect of the climate change on honey bee colonies in a temperate Mediterranean zone assessed through remote hive weight monitoring system in conjunction with exhaustive colonies assessment. Sci. Total Environ. 653: 1111-1119.
[https://doi.org/10.1016/j.scitotenv.2018.11.004]
-
Garibaldi, L. A., F. Requier, O. Rollin and G. K. Andersson. 2017. Towards an integrated species and habitat management of crop pollination. Curr. Opin. Insect Sci. 21: 105-114.
[https://doi.org/10.1016/j.cois.2017.05.016]
-
Garibaldi, L. A., L. G. Carvalheiro, S. D. Leonhardt, M. A. Aizen, B. R. Blaauw, R. Isaacs, M. Kuhlmann, D. Kleijn, A. M. Klein, C. Kremen, L. Morandin, J. Scheper and R. Winfree. 2014. From research to action: enhancing crop yield through wild pollinators. Front. Ecol. Environ. 12(8): 439-447.
[https://doi.org/10.1890/130330]
-
Giannini, T. C., W. F. Costa, G. D. Cordeiro, V. L. Imperatriz-Fonseca, A. M. Saraiva, J. Biesmeijer and L. A. Garibaldi. 2017. Projected climate change threatens pollinators and crop production in Brazil. PLoS One 12(8): e0182274.
[https://doi.org/10.1371/journal.pone.0182274]
-
Heldmaier, G. 1987. Temperature control in honey bee colonies. Bioscience 37(6): 395-399.
[https://doi.org/10.2307/1310562]
- Hill, D. S. 2012. The economic importance of insects. pp. 1-395. Springer Science & Business Media, London.
-
Howden, S. M., J. Soussana, F. N. Tubiello and H. Meinke. 2007. Adapting agriculture to climate change. Proc. Natl. Acad. Sci. U. S. A. 104(50): 19691-19696.
[https://doi.org/10.1073/pnas.0701890104]
-
Hristov, P., R. Shumkova, N. Palova and B. Neov. 2020. Factors associated with honey bee colony losses: A mini review. Vet. Sci. 7(4): 166.
[https://doi.org/10.3390/vetsci7040166]
-
Humphrey, J. A. C. and E. S. Dykes. 2008. Thermal energy conduction in a honey bee comb due to cell-heating bees. J. Theor. Biol. 250(1): 194-208.
[https://doi.org/10.1016/j.jtbi.2007.09.026]
- Jung, C. and J. H. Shin. 2022. Evaluation of crop production increase through insect pollination service in Korean agriculture. Korean J. Appl. Entomol. 61(1): 229-238.
-
Knoll, S., W. Pinna, A. Varcasia, A. Scala and M. G. Cappai. 2020. The honey bee (Apis mellifera L., 1758) and the seasonal adaptation of productions. Highlights on summer to winter transition and back to summer metabolic activity. A review. Livest. Sci. 235: 104011.
[https://doi.org/10.1016/j.livsci.2020.104011]
-
Lee, S. J., J. B. Lee, G. Choi, H. Y. Choi and C. R. Kwon. 2015. Analysis comparison of temperature change inside and outside of honey bee colonies according to the types of hive. J. Apic. 30(4): 253-257.
[https://doi.org/10.17519/apiculture.2015.11.30.4.253]
-
Losey, J. E. and M. Vaughan. 2006. The economic value of ecological services provided by insects. Bioscience 56(4): 311-323.
[https://doi.org/10.1641/0006-3568(2006)56[311:TEVOES]2.0.CO;2]
-
Monteleone, B., I. Borzi, B. Bonaccorso and M. Martina. 2023. Quantifying crop vulnerability to weather-related extreme events and climate change through vulnerability curves. Nat. Hazards 116(3): 2761-2796.
[https://doi.org/10.1007/s11069-022-05791-0]
-
Nelson, G. C., H. Valin, R. D. Sands, P. Havlik, H. Ahammad, D. Deryng, J. Elliott, S. Fujimori, T. Hasegawa, E. Heyhoe, P. Kyle, M. V. Lampe, H. Lotze-Campen, D. M. d’Croz, H. V. Meijl, D. V. D. Mensbrugghe, C. Muller, A. Popp, R. Robertson, S. Robinson, E. Schmid, C. Schmitz, A. Tabeau and D. Willenbockel. 2014. Climate change effects on agriculture: Economic responses to biophysical shocks. Proc. Natl. Acad. Sci. U. S. A. 111(9): 3274-3279.
[https://doi.org/10.1073/pnas.1222465110]
-
Neov, B., A. Georgieva, R. Shumkova, G. Radoslavov and P. Hristov. 2019. Biotic and abiotic factors associated with colonies mortalities of managed honey bee (Apis mellifera). Diversity-Basel 11(12): 237.
[https://doi.org/10.3390/d11120237]
-
Pauls, S. U., C. Nowak, M. Balint and M. Pfenninger. 2013. The impact of global climate change on genetic diversity within populations and species. Mol. Ecol. 22(4): 925-946.
[https://doi.org/10.1111/mec.12152]
-
Pecenka, J. R., L. L. Ingwell, C. H. Krupke and I. Kaplan. 2023. Implementing IPM in crop management simultaneously improves the health of managed bees and enhances the diversity of wild pollinator communities. Sci. Rep. 13(1): 11033.
[https://doi.org/10.1038/s41598-023-38053-5]
-
Peck, L. S. 2011. Organisms and responses to environmental change. Mar. Genom. 4(4): 237-243.
[https://doi.org/10.1016/j.margen.2011.07.001]
- R Core Team. 2023. R: A language and environment for statistical computing. R Foundation for Statistical Computing.
- Resh, V. H. and R. T. Carde. 2009. In encyclopedia of insects. pp. 220-227. Academic Press, Cambridge.
-
Rodriguez-Vasquez, S., A. A. G. Bejar, A. Romo-Chacon, J. Garcia-Hernandez, H. Gonzalez-Rios and J. A. Orozco-Avitia. 2024. Hive temperature regulation by honeybees in response to extreme conditions. Agrociencia 58(7): 1-15.
[https://doi.org/10.47163/agrociencia.v58i3.3082]
-
Rosenzweig, C., F. N. Tubiello, R. Goldberg, E. Mills and J. Bloomfield. 2002. Increased crop damage in the US from excess precipitation under climate change. Glob. Environ. Change 12(3): 197-202.
[https://doi.org/10.1016/S0959-3780(02)00008-0]
-
Rusmayandi, G., Indriyani, E. S. Hertini, M. A. K. Harahap and R. J. Nugroho. 2023. Climate change, agricultural production, food security, and livelihoods of rural communities in rural districts: A qualitative approach. West Sci. Interdiscip. Stud. 1(3): 102-110.
[https://doi.org/10.58812/wsis.v1i02.43]
-
Skendzic, S., M. Zovko, I. P. Zivkovic, V. Lesic and D. Lemic. 2021. The impact of climate change on agriculture insect pests. Insects 12(5): 440.
[https://doi.org/10.3390/insects12050440]
-
Stabentheiner, A., H. Kovac and R. Brodschneider. 2010. Honeybee colony thermoregulation - Regulatory mechanisms and contribution of individuals in dependence on age, location and thermal stress. PLoS One 5(1): e8967.
[https://doi.org/10.1371/journal.pone.0008967]
-
Thoms, C. A., K. C. Nelson, A. Kubas, N. Steinhauer, M. E. Wilson and D. vanEngelsdorp. 2019. Beekeeper stewardship, colony loss, and Varroa destructor management. Ambio 48: 1209-1218.
[https://doi.org/10.1007/s13280-018-1130-z]
-
Walther, G. 2010. Community and ecosystem responses to recent climate change. Philos. Trans. R. Soc. B-Biol. Sci. 365: 1549.
[https://doi.org/10.1098/rstb.2010.0021]
-
Yoon, H. J., K. Y. Lee, Y. B. Lee and M. Y. Lee. 2021. Current status of insect pollinators use for horticultural crops in Korea, 2020. J. Apic. 36(3): 111-123.
[https://doi.org/10.17519/apiculture.2021.09.36.3.111]
-
Zapata-Hernandez, G., M. Gajardo-Rojas, M. Calderon-Seguel, A. A. Munoz, K. P. Yanez, F. Requier, F. E. Fonturbel, P. I. Ormeno-Arriagada and H. Arrieta. 2024. Advances and knowledge gaps on climate change impacts on honey bees and beekeeping: A systematic review. Glob. Change Biol. 30(3): e17219.
[https://doi.org/10.1111/gcb.17219]
-
Ziegler, C., R. M. Ueda, T. Sinigaglia, F. Kreimeier and A. M. Souza. 2022. Correlation of climatic factors with the weight of an Apis mellifera beehive. Sustainability 14(9): 5302.
[https://doi.org/10.3390/su14095302]
-
Zilberman, D., X. Liu, D. Roland-Holst and D. Sunding. 2004. The economics of climate change in agriculture. Mitig. Adapt. Strateg. Glob. Chang. 9: 365-382.
[https://doi.org/10.1023/B:MITI.0000038844.72226.13]